Recovery
New energy choices for the future
Solar Power Generation
Yoshiaki Nakano
Director General and Professor at the Research Center for Advanced Science and Technology
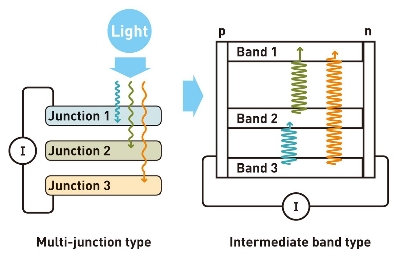
Structure of a solar cell with high conversion efficiency.
Sunlight is the most widely available natural energy source. Indeed the Sun provides the source for all forms of energy (except for nuclear power): fossil fuels were created by sunlight, and wind power, wave power, and biomass also represent energy created by sunlight. The total amount of energy delivered by the Sun is equivalent to the energy provided by about 50 million nuclear reactors. If we can harness that energy efficiently, we will never be short of energy.
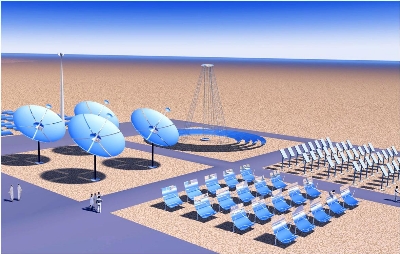
Illustration of solar power generation in the desert, the theme of the GS+I project.
Solar power generation is a technology that generates electrical power directly from sunlight, while solar thermal power generation is a similar but different technology that converts sunlight into thermal energy to generate electricity indirectly using turbines and by other conventional means. In solar power generation, solar cells play a core role in converting light energy directly into electrical energy. The biggest problem related to this method of power generation is variations in the amount of power generated, which depend on the weather and the length of the day and night. When such an unstable power source is connected to the current power system, other power generators need to operate in a pattern that compensates for the instability. This can severely affect the stability and efficiency of the entire system. This is the main reason why solar power generation has not been fully introduced. In Japan and other regions where the weather is not always clear, the operating rate of solar power generation systems would be low, which would in turn raise operating costs, making it difficult to introduce these systems on a large scale.
Before fully introducing solar power generation as a new energy source, it is essential to improve the conversion efficiency of solar cells, secure backup power sources, and develop large secondary batteries for short-term storage, as well as to develop technologies that can store solar energy temporarily or for a long period of time on a medium- to long-term basis. It is also necessary to further foster the introduction and spread of solar power generation in low-latitude desert areas where solar energy can be obtained at the lowest cost. To this end, the University of Tokyo has been commissioned by the New Energy and Industrial Technology Development Organization (NEDO) to research and develop innovative solar power generation technologies. Accordingly, the University has been conducting research and development on highly efficient next-generation solar cells, while launching a project to establish a sustainable global energy system based on the use of solar energy (“Endowed Chair for Global Solar+ Initiative”: GS+I) toward the large-scale introduction of solar power generation under international cooperation.
Offshore Wind Power
Takeshi Ishihara
Professor, Graduate School of Engineering
Some countries have begun constructing large-scale offshore wind farms with ambitious targets, because offshore winds are strong and less turbulence, and there is an extensive space for the construction of wind farms on the sea, where there will be few environmental problems related to landscape and noise. The installed capacity of offshore wind power reached 2.96 GW in Europe in 2010, showing an annual growth rate of 51%. The world’s largest offshore wind farm now under construction will have a capacity of 680MW, almost the same as a nuclear power plant. In Japan, seven 2MW wind turbines were installed offshore near Kamisu City, Ibaraki Prefecture in 2009 to produce electricity to supply around 7,000 households.
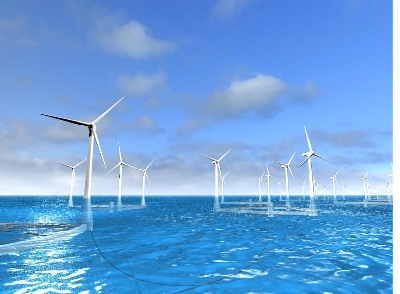
Architectural rendering of a floating offshore wind farm.
According to a survey conducted by the University of Tokyo in 2006, the theoretical wind potential available in Kanto coastal region is almost same as the annual power production by the Tokyo Electric Power Company in 2005, and this implies that large offshore wind farms can be constructed in the Pacific coastal region where wind conditions are good. Japan’s coastal waters are deep in comparison with Europe, and it is therefore necessary to use floating support structures in these areas. The University organized a joint research project on floating wind turbine systems with electric power companies, wind turbine and ship manufacturers and construction companies in Japan to propose semisubmersible, SPAR and TLP floaters and to promote a national R&D project for the practical use of these structures. Figure 1 shows the architectural rendering of a floating offshore wind farm.
Wind energy is resource without CO2 emission and can be obtained domestically, which differs from uranium and fossil fuels imported from overseas. Wind energy has also an advantage in cost and could have a significant effect on industry promotion and job creation (about 15,000 jobs/GW). As of the end of 2010, the installed capacity of wind turbines around the world totals 194.39 GW and investments in wind energy industry account for one-fifth of all investments for the electrical equipments. The wind power market is now seven trillion yen. The cost of wind power is a little higher than that of thermal power. However, fossil fuels for the thermal power accounts for 60% of the electrical production costs, while almost 100% of the money for wind power will be used at home because Japan has whole technologies. It is believed that the full-scale penetration of wind power in Japan will contribute to solving power shortage problems and significantly help Tohoku area to recover from the disaster by the Great East Japan Earthquake.
Biofuel
Shigeyuki Kawano
Professor, Graduate School of Frontier Sciences
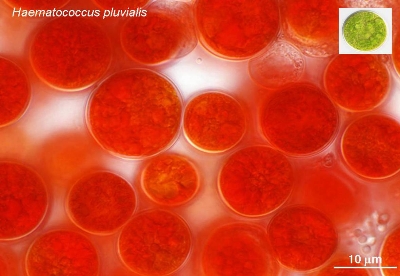
Haematococcus. This species, which is originally green, becomes red after accumulating astaxanthin when cultivated under strong light. Astaxanthin can be used as food coloring for chicken’s eggs and trout as well as antioxidant agent. The oil contained by the substance could also be used as a biofuel. There are also many other high-potential species that produce copious amounts of oil.
Although I had been feeling gloomy after the accident at the nuclear power station in Fukushima, I felt better after I heard some good news from France: a leading European aircraft manufacturer (EADS) announced at the Paris Air Show that jointly with Japan they were developing a supersonic airplane that could fly from Tokyo to Paris in two and a half hours. Its greatest feature is that the Zero Emission Hypersonic Transportation (Zehst) plane will not emit CO2 because it uses biofuel derived from seaweed. A prototype will be completed by 2020 and commercial operation will start by 2050; it is expected that algae-derived biofuel will also be in wide use by that time (Table 4).
In recent years, against the backdrop of spikes in crude oil prices, global warming, and the use of cereals in the production of bioethanol, both public and private organizations across the world are investing in research into biofuel derived from seaweeds and microalgae. There are high expectations for biofuel, as the case of the Zehst airplane shows. Biofuel research has progressed considerably. The first-generation biofuel (bioethanol, which was developed despite a range of problems) has been successfully developed, and speedy efforts have been made to put the second-generation biofuel, derived from camelina, jatropha and algae, into practical use. Compared with other plants, the productivity per area for algae is excellent, and it can be cultivated even in areas unsuitable for agriculture, thus not leading to a decrease in the farmland available for food crops. Algae are therefore high among the potential candidates for biofuel, and we are able to choose the most productive algal species for the local environment from among a variety of genetic resources (figure).
Item | Market size | Remarks |
---|---|---|
Biofuel | 128 to 1,105 billion yen | World market (forecast for 2015 to 2020)1 |
Laver | 300 billion yen | About 30,000 tons2 |
Kelp | 240 billion yen | Domestic market (on a retail basis)2 |
Wakame seaweed | 50 billion yen | Mostly farmed (97% domestic production and 100% imports)2 |
Chlorella | 30 billion yen | About 1,000 tons3 |
Astaxanthin | 10 billion yen | Materials: One ton (up 20% year-on-year)3 |
Fucoidan | 10 billion yen | Domestic market (forecast for 2008)3 |
Euglena | 5 billion yen | Domestic market (forecast for 2015)4 |
Spirulina | 800 million yen | About 150 tons/year; 5,000 yen/kg (surveyed in 2009) |
Fucoxanthin | 200 million yen | About 0.5 ton/year; 150,000 to 300,000 yen/kg (surveyed in 2008)3 |
1. Source: Global information, Inc. 2. Source: Sato, J. (2002) Processed seaweeds in “The situation of phycology at the beginning of the 21st century” edited by T. Hori, M. Ono, and T. Horiguchi, The Japanese Society of Phycology (Yamagata), pp 140–142. 3. Source: Genryobank 4. Source: SankeiBiz November 3, 2010 |
Ocean Energy
Takeshi Kinoshita
Professor, Institute of Industrial Science
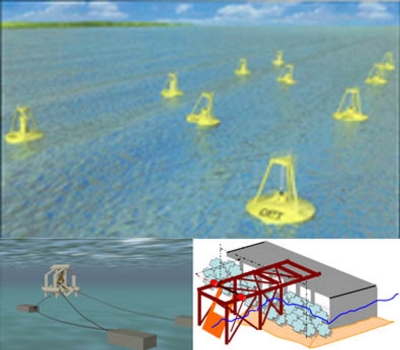
Wave farm and coastal wave power generation facilities (example).
The amount of energy that could be derived from wave power, ocean currents, and thermal difference in the seas surrounding Japan (to a depth not exceeding 100 m and within 30 km from the coast) is equivalent to the amount of electricity generated by 482 nuclear reactors (NEDO, 2010). Including areas to a depth of between 100 and 200 m, which would be the main areas for the floating wind farms that are being developed competitively across the world, the amount of ocean energy available will increase by several fold. Ocean energy is readily available, especially in the service areas of the Tokyo Electric Power Company, Okinawa Electric Power Company, Tohoku Electric Power Company, and Hokkaido Electric Power Company.
The economic efficiency of ocean energy is expected to equal other forms of new energy by 2020, as shown in Table 5. Before putting ocean energy into commercial use, demonstration tests must be carried out in actual sea areas, and Japan is behind other countries in this regard. Japan, however, has a lot of sea areas where in two to three years we can carry out demonstration tests in the commercial use of ocean energy and make Japan a frontrunner in the field. As part of our efforts to support Japan’s recovery from the March disaster, we are proposing an initiative to help Fukushima recover from the disaster through the use of ocean energy. Specific to this initiative, which will be implemented in cooperation with local communities, we will establish a demonstration test site and launch commercial operation of an offshore energy farm. Through this initiative, we aim to change the negative image of Fukushima caused by the accident at the nuclear power station to a positive one by making it a model area for building up local industries based on the use of ocean energy.
Type | Present | 2015 | 2020 | 2030 |
---|---|---|---|---|
Solar power | (48 yen/kWh) | 23 yen/kWh | 14 yen/kWh | 7 yen/kWh |
Land wind power | 9–15 yen/kWh | 7–11 yen/kWh | 5–8 yen/kWh | |
Offshore wind power | (9–15 yen/kWh) | 12–17 yen/kWh | 8–11 yen/kWh | |
Solar thermal power | 13–30 yen/kWh | 10–15 yen/kWh | 5–17 yen/kWh | |
Wave power | (30–50 yen/kWh) | –40 yen/kWh | –20 yen/kWh | 5–10 yen/kWh |
Ocean thermal energy conversion | 40–6 yen/kWh | 15–25 yen/kWh | 8–13 yen/kWh | |
Source: NEDO’s technological report on renewable energy (2010) |
Demonstration test site | Commercialization (Round 1) | Commercialization (Round 2) | |
---|---|---|---|
Period | 2011-2015 | -2020 | 2011-2015 |
Target | Open an ocean energy demonstration test site by 2013 (Japanese version of EMEC) Complete development for commercialization (first stage) and establish the infrastructure by 2015 |
6 GW in Fukushima: Offshore wind power generation: 5GW Wave power generation: 1GW (Equivalent to the amount of electricity generated by three nuclear reactors) |
50GW across Japan (Equivalent to the amount of electricity generated by 25 nuclear reactors) |
Cost of establishing peripheral equipment such as undersea power cables | 5 billion yen | 400 to 500 billion yen | Private sector |
Local revitalization measures | Also carry out demonstration tests on revitalization measures implemented for fisheries and tourism | Introduce highly effective measures | Across the country |
Methane Hydrate
Jun Matsushima
Associate Professor, Graduate School of Engineering
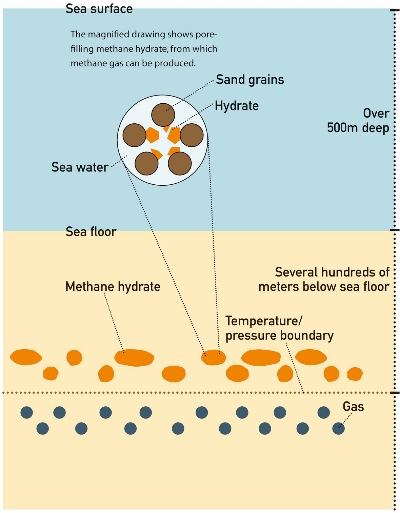
Illustration of a methane hydrate-bearing layer.
Methane hydrate is attracting much attention as a domestic energy resource for the future. Methane hydrate is the main component of natural gas and is a solid substance similar to ice, in which methane is trapped within a cage structure composed of water molecules under high-pressure and low-temperature conditions. On putting a lighter close to the substance, the cage structure is broken and methane begins to burn, and so methane hydrate is also known as “fiery ice.” In nature, methane hydrate exists in permafrostareas and in deep water sedimentary layers. It is worth noting that the total amount of the substance is estimated to be more than half of all the organic carbons present on the Earth. The substance is estimated to exist in large amounts also around offshore Japan. It originates from organic matter which is abundantly supplied from steep mountains on land. Methane hydrate is indeed a blessing of nature resulting from Japan’s natural environment in which we have both steep mountains and a deep ocean floor. Not only Japan, but also the United States, Canada, India, South Korea, China, and other countries are implementing projects to use this substance as a resource towards commercial production by around 2020. The biggest technological challenge is to derive methane gas from the methane hydrate located underground, and it is said that the most effective means of achieving this is the depressurization-induced dissociation method in the underground methane hydrate-bearing layers to gasify the substance. Production tests conducted in permafrost areas in Canada have suggested that this method will be effective. In Japan the world’s first ocean production test is planned to be conducted in and after 2012. I believe it is important for us to realize that because of Japan’s natural environment, which was created by dynamic movements in the Earth’s crust, we can utilize methane hydrate to develop a new local industry in Japan, which currently depends on imported energy and resources.
Fuel Cell/Battery System
Atsushi Tsutsumi
Professor, Institute of Industrial Science; Director, Collaborative Research Center for Energy Engineering
The Collaborative Research Center for Energy Engineering (CEE) was established in January 2008 to develop innovative energy technologies. At the center we are working to indentify and clarify a range of problems existing in the energy field, develop energy technologies, analyze energy scenarios, and plan energy strategies. In response to the March 11 disaster, we quickly established a website to make available information useful for saving power, while holding symposiums on the future supply and demand of energy. In this way, we are introducing measures to contribute to Japan’s recovery from the disaster.
After the disaster, power shortages became a great social concern and people are now paying more attention to power generation using renewable energy such as solar cells, wind power, biomass, wave power, and geothermal heat. The outputs of renewable energy, however, vary by time of day, and the demand for power also changes rapidly by time of day. To level off these changes in supply and demand, it is critical that we develop energy storage technologies. In particular, to promote the use of natural energy, it is important to build systems that combine distributed power sources such as solar cells and fuel cells with secondary batteries.
The development of batteries has been progressing not only for the storage of electricity but also for use in PCs and mobile devices such as cell phones as well as in electric vehicles (EVs). The figure below shows the energy density and output density of various power storage devices. Compared with fuel cells, secondary batteries such as lithium ion batteries have an output density that is larger by one digit and an energy density that is smaller by two digits. Batteries used for the storage of electricity and in EVs need to have the same energy density as a fuel cell while having an even larger output density. At the CEE, electrode reactions in fuel cells are being made at a two-phase interface instead of a three-phase interface by dividing the reactions into hydrogen absorption and cell reaction, with a view to developing a fuel cell/battery (FCB) system that has an output density and energy density comparable to the output density of secondary batteries and the energy density of fuel cells. In helping Japan to recover from the disaster, it is important for the University of Tokyo as a whole to steadily develop innovative energy technologies while continuing to develop human resources and disseminating relevant information.